This story was originally posted by the Graduate School
Five outstanding scholars, including Physics PhD student Jimena González, are joining the UW–Madison chapter of the national Edward Alexander Bouchet Graduate Honor Society this academic year.
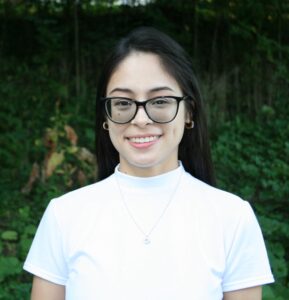
The Bouchet Society commemorates the first person of African heritage to earn a PhD in the United States. Edward A. Bouchet earned a PhD in Physics from Yale University in 1876. Since then, the Bouchet Society has continued to uphold Dr. Bouchet’s legacy.
“The 2024 Bouchet inductees are making key contributions in their disciplines, as well as to the research, education, and outreach missions of our campus. They truly embody the Wisconsin Idea and are exemplary in every way,” said Abbey Thompson, assistant dean for diversity, inclusion, and funding in the Graduate School.
The Bouchet Society serves as a network for scholars that uphold the same personal and academic excellence that Dr. Bouchet demonstrated. Inductees to the UW–Madison Chapter of the Bouchet Society also join a national network with 20 chapters across the U.S. and are invited to present their work at the Bouchet Annual Conference at Yale University, where the scholars further create connections and community within the national Bouchet Society.
The UW–Madison Division of Diversity, Equity, and Educational Achievement supports each inductee with a professional development grant.
González is a physics PhD candidate specializing in observational cosmology. Her research centers on searching and characterizing strong gravitational lenses in the Dark Energy Survey. These rare astronomical systems can appear as long curved arcs of light surrounding a galaxy. Strong gravitational lenses offer a unique probe for studying dark energy, the driving force behind the universe’s accelerating expansion and, consequently, a pivotal factor in determining its ultimate fate.
During her graduate program, Jimena has received the Albert R. Erwin, Jr. & Casey Durandet Award and the Firminhac Fellowship from the Department of Physics. Additionally, she was honored with the 2023 Open Science Grid David Swanson Award for her outstanding implementation of High-Throughput Computing to advance her research. Jimena has contributed as a co-author to multiple publications within the field of strong gravitational lensing and has presented her work at various conferences. In addition to her academic achievements, Jimena has actively engaged in outreach programs. Notably, she was selected as a finalist at the 2021 UW–Madison Three Minute Thesis Competition and secured a winning entry in the 2023 Cool Science Image Contest. Her commitment to science communication extends to a contribution in a Cosmology chapter in the book AI for Physics. Jimena has also led a citizen science project that invites individuals from all around the world to inspect astronomical images to identify strong gravitational lenses. Jimena obtained her bachelor’s degree in physics at the Universidad de los Andes, where she was awarded the “Quiero Estudiar” scholarship.